In the atmosphere, both temperature and pressure decrease with altitude, and have conflicting effects upon density. However, the fairly rapid drop in pressure as altitude is increased usually has the dominating effect. Hence, density can be expected to decrease with altitude.
The pilot's handbook of aeronautical knowledge introduces pilots to the broad spectrum of aeronautical knowledge that will be needed as they progress in their pilot training.
Tuesday, September 30, 2008
Effect of Temperature on Density
In the atmosphere, both temperature and pressure decrease with altitude, and have conflicting effects upon density. However, the fairly rapid drop in pressure as altitude is increased usually has the dominating effect. Hence, density can be expected to decrease with altitude.
Monday, September 29, 2008
Effects of Pressure on Density
Sunday, September 28, 2008
Atmospheric Pressure
Though air is very light, it has mass and is affected by the attraction of gravity. Therefore, like any other substance, it has weight, and because of its weight, it has force. Since it is a fluid substance, this force is exerted equally in all directions, and its effect on bodies within the air is called pressure. Under standard conditions at sea level, the average pressure exerted on the human body by the weight of the atmosphere around it is approximately 14.7 lb./in. The density of air has significant effects on the airplane’s capability. As air becomes less dense, it reduces (1) power because the engine takes in less air, (2) thrust because the propeller is less efficient in thin air, and (3) lift because the thin air exerts less force on the airfoils.
Saturday, September 27, 2008
Structure of the Atmosphere
Air, like any other fluid, is able to flow and change its shape when subjected to even minute pressures because of the lack of strong molecular cohesion. For example, gas will completely fill any container into which it is placed, expanding or contracting to adjust its shape to the limits of the container.
The atmosphere is composed of 78 percent nitrogen, 21 percent oxygen, and 1 percent other gases, such as argon or helium. As some of these elements are heavier than others, there is a natural tendency of these heavier elements, such as oxygen, to settle to the surface of the earth, while the lighter elements are lifted up to the region of higher altitude. This explains why most of the oxygen is contained below 35,000 feet altitude.
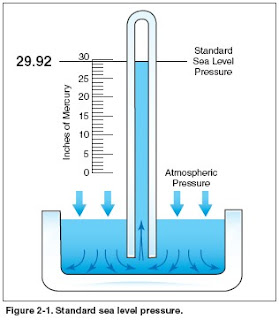
Friday, September 26, 2008
The Powerplant
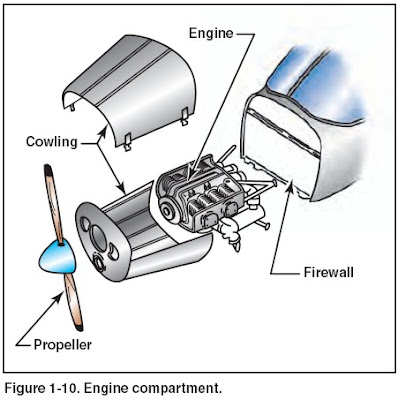
Nacelle—A streamlined enclosure on an aircraft in which an engine is mounted. On multiengine propeller-driven airplanes, the nacelle is normally mounted on the leading edge of the wing.
Thursday, September 25, 2008
Landing Gear
most common type of landing gear consists of wheels, but airplanes can also be equipped with floats for water operations, or skis for landing on snow. [Figure 1-9]
The landing gear consists of three wheels—two main wheels and a third wheel positioned either at the front or rear of the airplane. Landing gear employing a rearmounted wheel is called conventional landing gear. Airplanes with conventional landing gear are sometimes referred to as tailwheel airplanes. When the third wheel is located on the nose, it is called a nosewheel, and the design is referred to as a tricycle gear. A steerable nosewheel or tailwheel permits the airplane to be controlled throughout all operations while on the ground.
Wednesday, September 24, 2008
Empennage
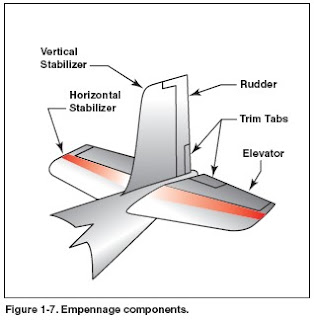
A second type of empennage design does not require an elevator. Instead, it incorporates a one-piece horizontal stabilizer that pivots from a central hinge point. This type of design is called a stabilator, and is moved using the control wheel, just as you would the elevator. For example, when you pull back on the control wheel, the stabilator pivots so the trailing edge moves up. This increases the aerodynamic tail load and causes the nose of the airplane to move up. Stabilators have an antiservo tab extending across their trailing edge. [Figure 1-8]
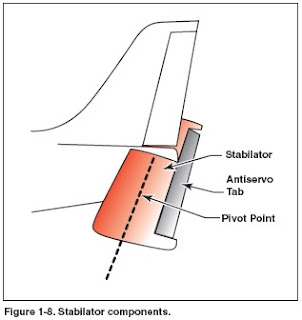
The rudder is attached to the back of the vertical stabilizer. During flight, it is used to move the airplane’s nose left and right. The rudder is used in combination with the ailerons for turns during flight. The elevator, which is attached to the back of the horizontal stabilizer, is used to move the nose of the airplane up and down during flight.
Trim tabs are small, movable portions of the trailing edge of the control surface. These movable trim tabs, which are controlled from the cockpit, reduce control pressures. Trim tabs may be installed on the ailerons, the rudder, and/or the elevator.
Empennage—The section of the airplane that consists of the vertical stabilizer, the horizontal stabilizer, and the associated control surfaces.
Tuesday, September 23, 2008
Wings
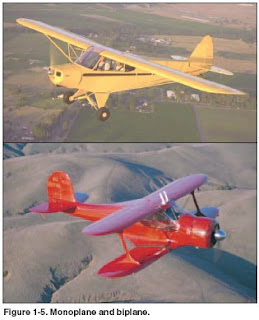
Many high-wing airplanes have external braces, or wing struts, which transmit the flight and landing loads through the struts to the main fuselage structure. Since the wing struts are usually attached approximately halfway out on the wing, this type of wing structure is called semi-cantilever. A few high-wing and most low-wing airplanes have a full cantilever wing designed to carry the loads without external struts.
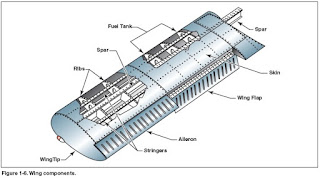
Attached to the rear, or trailing, edges of the wings are two types of control surfaces referred to as ailerons and flaps. Ailerons extend from about the midpoint of each wing outward toward the tip and move in opposite directions to create aerodynamic forces that cause the airplane to roll. Flaps extend outward from the fuselage to near the midpoint of each wing. The flaps are normally flush with the wing’s surface during cruising flight. When extended, the flaps move simultaneously downward to increase the lifting force of the wing for takeoffs and landings.
Airfoil—An airfoil is any surface, such as a wing, propeller, rudder, or even a trim tab, which provides aerodynamic force when it interacts with a moving stream of air. Monoplane—An airplane that has only one main lifting surface or wing, usually divided into two parts by the fuselage.
Biplane—An airplane that has two main airfoil surfaces or wings on each side of the fuselage, one placed above the other.
Monday, September 22, 2008
Fuselage
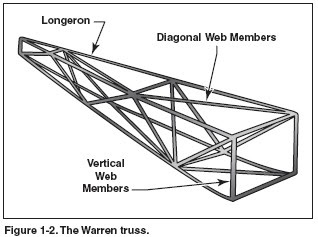
Construction of the Warren truss features longerons, as well as diagonal and vertical web members. To reduce weight, small airplanes generally utilize aluminum alloy tubing, which may be riveted or bolted into one piece with cross-bracing members.
As technology progressed, aircraft designers began to enclose the truss members to streamline the airplane and improve performance. This was originally accomplished with cloth fabric, which eventually gave way to lightweight metals such as aluminum. In some cases, the outside skin can support all or a major portion of the flight loads. Most modern aircraft use a form of this stressed skin structure known as monocoque or semimonocoque construction
The monocoque design uses stressed skin to support almost all imposed loads. This structure can be very strong but cannot tolerate dents or deformation of the surface. This characteristic is easily demonstrated by a thin aluminum beverage can. You can exert considerable force to the ends of the can without causing any damage.
Truss—A fuselage design made up of supporting structural members that resist deformation by applied loads.
Monocoque—A shell-like fuselage design in which the stressed outer skin is used to support the majority of imposed stresses. Monocoque fuselage design may include bulkheads but not stringers.
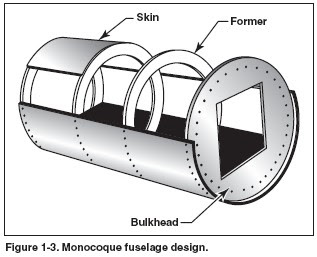
Since no bracing members are present, the skin must be strong enough to keep the fuselage rigid. Thus, a significant problem involved in monocoque construction is maintaining enough strength while keeping the weight within allowable limits. Due to the limitations of the monocoque design, a semi-monocoque structure is used on many of today’s aircraft.
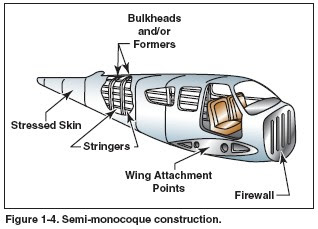
Semi-Monocoque—A fuselage design that includes a substructure of bulkheads and/or formers, along with stringers, to support flight loads and stresses imposed on the fuselage.
On single-engine airplanes, the engine is usually attached to the front of the fuselage. There is a fireproof partition between the rear of the engine and the cockpit or cabin to protect the pilot and passengers from accidental engine fires. This partition is called a firewall and is usually made of heat-resistant material such as stainless steel.
Sunday, September 21, 2008
Major Component of Aircraft Structure
Aircraft—A device that is used for flight in the air.
Airplane—An engine-driven, fixed-wing aircraft heavier than air that is supported in flight by the dynamic reaction of air against its wings.
MAJOR COMPONENTS
Although airplanes are designed for a variety of purposes, most of them have the same major components. The overall characteristics are largely determined by the original design objectives. Most airplane structures include a fuselage, wings, an empennage, landing gear, and a powerplant. [Figure 1-1]
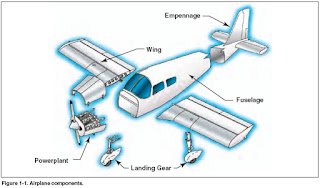
Thursday, September 18, 2008
Basic Weight and Balance Equation
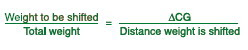

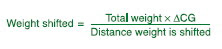


This same problem can also be solved by using this basic equation:


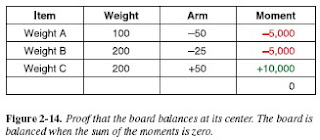
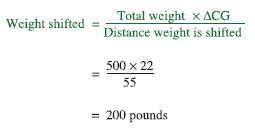
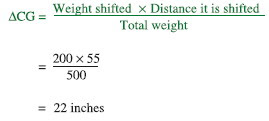
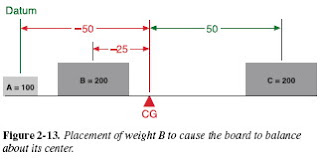
Wednesday, September 17, 2008
Shifting the CG
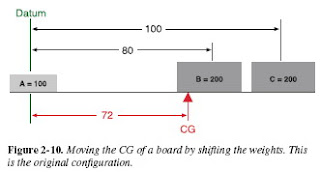
Solution by Chart
The CG of a board can be moved by shifting the weights as demonstrated in Figure 2-10. As the board is loaded, it balances at a point 72 inches from the CG of weight A. [Figure 2-11]
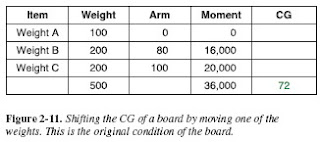
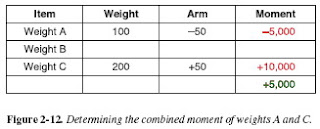
Determine the arm of weight B by dividing its moment, -5,000 lb-in, by its weight of 200 pounds. Its arm is -25 inches.
To balance the board at its center, weight B will have to be placed so its CG is 25 inches to the left of the center of the board, as in Figure 2-13.
Tuesday, September 16, 2008
Determining the CG
One of the easiest ways to understand weight and balance is to consider a board with weights placed at various locations. We can determine the CG of the board and observe the way the CG changes as the weights are moved.
The CG of a board like the one in Figure 2-4 may be determined by using these four steps:
- Measure the arm of each weight in inches from the datum.
- Multiply each arm by its weight in pounds to determine the moment in pound-inches of each weight.
- Determine the total of all weights and of all the moments. Disregard the weight of the board.
- Divide the total moment by the total weight to determine the CG in inches from the datum.
In Figure 2-4, the board has three weights, and the datum is located 50 inches to the left of the CG of weight A. Determine the CG by making a chart like the one in Figure 2-5. As noted in Figure 2-5, A weighs 100 pounds and is 50 inches from the datum: B weighs 100 pounds and is 90 inches from the datum; C weighs 200 pounds and is 150 inches from the datum. Thus the total of the three weights is 400 pounds, and the total moment is 44,000 lb-in.
Determine the CG by dividing the total moment by the total weight.
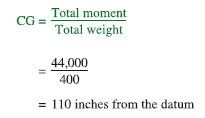
To prove this is the correct CG, move the datum to a location 110 to the right of the original datum and determine the arm of each weight from this new datum, as in Figure 2-6. Then make a new chart similar to the one in Figure 2-7. If the CG is correct, the sum of the moments will be zero.
The new arm of weight A is 110 - 50 = 60 inches, and since this weight is to the left of the datum, its arm is negative, or -60 inches. The new arm of weight B is 110 - 90 = 20 inches, and it is also to the left of the datum, so it is - 20; the new arm of weight C is 150 - 110 = 40 inches. It is to the right of the datum and is therefore positive.The board is balanced when the sum of the moments is zero. The location of the datum used for determining the arms of the weights is not important; it can be anywhere. But all of the measurements must be made from the same datum location.
Determining the CG of an airplane is done in the same way as determining the CG of the board in the previous example. [Figure 2-8] Prepare the airplane for weighing and place it on three scales. All tare weight, that is, the weight of any chocks or devices used to hold the aircraft on the scales, is subtracted from the scale reading, and the net weight from each wheel weigh point is entered on the chart like the one in Figure 2-9. The arms of the weighing points are specified in the Type Certificate Data Sheet (TCDS) for the airplane in terms of stations, which are distances in inches from the datum.
Tare weight also includes items used to level the aircraft.
The empty weight of this aircraft is 5,862 pounds. Its EWCG, determined by dividing the total moment by the total weight, is located at fuselage station 201.1. This is 201.1 inches behind the datum.
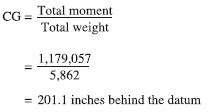
Monday, September 15, 2008
The Law of the Lever
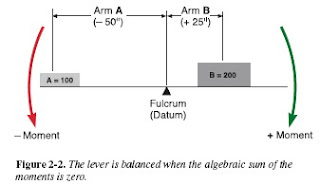
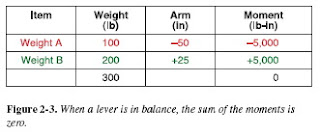
Sunday, September 14, 2008
Aircraft Arms, Weight, and Moments
The manufacturer establishes the maximum weight and range allowed for the CG, as measured in inches from the reference plane called the datum. Some manufacturers specify this range as measured in percentage of the mean aerodynamic chord (MAC), the leading edge of which is located a specified distance from the datum.
The datum may be located anywhere the manufacturer chooses; it is often the leading edge of the wing or some specific distance from an easily identified location. One popular location for the datum is a specified distance forward of the aircraft, measured in inches from some point, such as the nose of the aircraft, or the leading edge of the wing, or the engine firewall.
The datum of some helicopters is the center of the rotor mast, but this location causes some arms to be positive and others negative. To simplify weight and balance computations, most modern helicopters, like airplanes, have the datum located at the nose of the aircraft or a specified distance ahead of it.
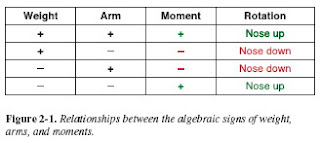
Saturday, September 13, 2008
Weight and Balance Theory
- The total weight of the aircraft must be no greater than the maximum weight allowed by the FAA for the particular make and model of the aircraft.
- The center of gravity, or the point at which all of the weight of the aircraft is considered to be concentrated, must be maintained within the allowable range for the operational weight of the aircraft.
Weight Control for Aircraft other than Fixed and Rotorwing
These aircraft typically do not specify either an empty weight center of gravity or a center of gravity range. They require only a certified or approved maximum weight.
To understand why this is so, a look at how flight control is achieved is helpful.
As an example, airplanes and WSC aircraft both control flight under the influence of the same four forces (lift, gravity, thrust, and drag), and around the same three axes (pitch, yaw, and roll). However, each aircraft accomplishes this control in a very different manner. This difference helps explain why the fixed-wing airplane requires an established weight and a known center of gravity, whereas the WSC aircraft only requires the known weight.
The fixed-wing airplane has moveable controls that alter the lift on various airfoil surfaces to vary pitch, roll, and yaw. These changes in lift, in turn, change the characteristics of the flight parameters. Weight normally decreases in flight due to fuel consumption, and the airplane center of gravity changes with this weight reduction. An airplane utilizes its variable flight controls to compensate and maintain controllability through the various flight modes and as the center of gravity changes. An airplane has a center of gravity range or envelope within which it must remain if the flight controls are to remain effective and the airplane safely operated.
The WSC aircraft has a relatively set platform wing without a tail. The pilot, achieves control by shifting weight. In the design of this aircraft, the weight of the airframe and its payload is attached to the wing at a single point in a pendulous arrangement. The pilot through the flight controls, controls the arm of this pendulum and thereby controls the aircraft. When a change in flight parameter is desired, the pilot displaces the aircraft’s weight in the appropriate distance and direction. This change momentarily disrupts the equilibrium between the four forces acting on the aircraft. The wing, due to its inherent stability, then moves appropriately to re-establish the desired relationship between these forces. This happens by the wing flexing and altering its shape. As the shape is changed, lift is varied at different points on the wing to achieve the desired flight parameters.
The flight controls primarily affect the pitch-and-roll axis. Since there is no vertical tail plane, minimal or no ability exists to directly control yaw. However, unlike the airplane, the center of gravity experienced by the wing remains constant. Since the weight of the airframe acts through the single point (wing attach point), the range over which the weight may act is fixed at the pendulum arm or length. Even though the weight decreases as fuel is consumed, the weight remains focused at the wing attach point. Most importantly, because the range is fixed, the need to establish a calculated range is not required.
The powered parachute also belongs to the pendulumstyle aircraft. Its airframe center of gravity is fixed at the pendulum attach point. It is more limited in controllability than the WSC aircraft because it lacks an aerodynamic pitch control. Pitch (and lift) control is primarily a function of the power control. Increased power results in increased lift; cruise power amounts to level flight; decreased power causes a descent. Due to this characteristic, the aircraft is basically a one-air speed aircraft. Once again, because the center of gravity is fixed at the attach point to the wing, there can be no center of gravity range.
Roll control on a powered parachute is achieved by changing the shape of the wing. The change is achieved by varying the length of steering lines attached to the outboard trailing edges of the wing. The trailing edge of the parachute is pulled down slightly on one side or the other to create increased drag along that side. This change in drag creates roll and yaw, permitting the aircraft to be steered.
The balloon is controlled by the pilot only in the vertical dimension; this is in contrast to all other aircraft. He or she achieves this control through the use of lift and weight. Wind provides all other movement. The center of gravity of the gondola remains constant beneath the balloon envelope. As in WSC and powered-parachute aircraft, there is no center of gravity limitation.
Aircraft can perform safely and achieve their designed efficiency only when they are operated and maintained in the way their designers intended. This safety and efficiency is determined to a large degree by holding the aircraft’s weight and balance parameters within the limits specified for its design. The remainder of this handbook describes the way in which this is done.
Friday, September 12, 2008
Stability and Balance Control
The CG is the point at which the total weight of the aircraft is assumed to be concentrated, and the CG must be located within specific limits for safe flight. Both lateral and longitudinal balance are important, but the prime concern is longitudinal balance; that is, the location of the CG along the longitudinal or lengthwise axis.
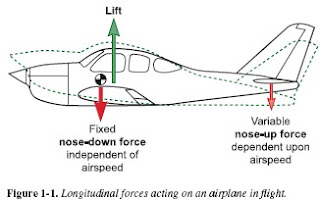
If a rising air current should cause the nose to pitch up, the airplane will slow down and the downward force on the tail will decrease. The weight concentrated at the CG will pull the nose back down. If the nose should drop in flight, the airspeed will increase and the increased downward tail load will bring the nose back up to level flight.
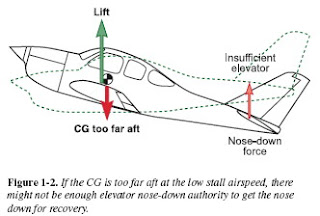
If the CG is too far forward, the downward tail load will have to be increased to maintain level flight. This increased tail load has the same effect as carrying additional weight; the aircraft will have to fly at a higher angle of attack, and drag will increase.
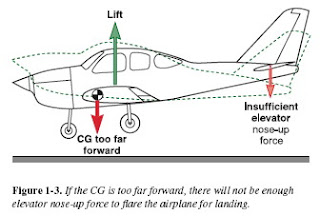
The basic aircraft design assumes that lateral symmetry exists. For each item of weight added to the left of the centerline of the aircraft (also known as buttock line zero, or BL-0), there is generally an equal weight at a corresponding location on the right.
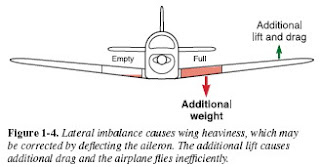
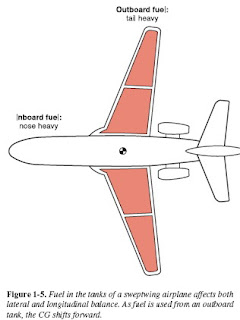
Sweptwing airplanes are more critical due to fuel imbalance because as the fuel is used from the outboard tanks, the CG shifts forward, and as it is used from the inboard tanks, the CG shifts aft. [Figure 1-5] For this reason, fuel-use scheduling in sweptwing airplanes operation is critical.
Saturday, September 6, 2008
Weight Changes
Effects of Weight
Most modern aircraft are so designed that if all seats are occupied, all baggage allowed by the baggage compartment is carried, and all of the fuel tanks are full, the aircraft will be grossly overloaded. This type of design requires the pilot to give great consideration to the requirements of the trip. If maximum range is required, occupants or baggage must be left behind, or if the maximum load must be carried, the range, dictated by the amount of fuel on board, must be reduced.
Some of the problems caused by overloading an aircraft
are:
- the aircraft will need a higher takeoff speed, whichresults in a longer takeoff run.
- both the rate and angle of climb will be reduced.
- the service ceiling will be lowered.
- the cruising speed will be reduced.
- the cruising range will be shortened.
- maneuverability will be decreased.
- a longer landing roll will be required because the landing speed will be higher.
- excessive loads will be imposed on the structure, especially the landing gear.
The POH or AFM includes tables or charts that give the pilot an indication of the performance expected for any weight. An important part of careful preflight planning includes a check of these charts to determine the aircraft is loaded so the proposed flight can be safely made.
Friday, September 5, 2008
Weight Control
When an aircraft is designed, it is made as light as the required structural strength will allow, and the wings or rotors are designed to support the maximum allowable weight. When the weight of an aircraft is increased, the wings or rotors must produce additional lift and the structure must support not only the additional static loads, but also the dynamic loads imposed by flight maneuvers. For example, the wings of a 3,000-pound airplane must support 3,000 pounds in level flight, but when the airplane is turned smoothly and sharply using a bank angle of 60°, the dynamic load requires the wings to support twice this, or 6,000 pounds.
Severe uncoordinated maneuvers or flight into turbulence can impose dynamic loads on the structure great enough 1– to cause failure. In accordance with Title 14 of the Code of Federal Regulations (14 CFR) part 23, the structure of a normal category airplane must be strong enough to sustain a load factor of 3.8 times its weight. That is, every pound of weight added to an aircraft requires that the structure be strong enough to support an additional 3.8 pounds. An aircraft operated in the utility category must sustain a load factor of 4.4, and acrobatic category aircraft must be strong enough to withstand 6.0 times their weight.
The lift produced by a wing is determined by its airfoil shape, angle of attack, speed through the air, and the air density. When an aircraft takes off from an airport with a high density altitude, it must accelerate to a speed faster than would be required at sea level to produce enough lift to allow takeoff; therefore, a longer takeoff run is necessary. The distance needed may be longer than the available runway. When operating from a high-density altitude airport, the Pilot’s Operating Handbook (POH) or Airplane Flight Manual (AFM) must be consulted to determine the maximum weight allowed for the aircraft under the conditions of altitude, temperature, wind, and runway conditions.
Thursday, September 4, 2008
Weight and Balance Control
The pilot in command of the aircraft has the responsibility on every flight to know the maximum allowable weight of the aircraft and its CG limits. This allows the pilot to determine on the preflight inspection that the aircraft is loaded in such a way that the CG is within the allowable limits.
Tuesday, September 2, 2008
FLIGHT PLANNING
ASSEMBLING NECESSARY MATERIAL
The pilot should collect the necessary material well before the flight. An appropriate current sectional chart and charts for areas adjoining the flight route should be among this material if the route of flight is near the border of a chart.
Additional equipment should include a flight computer or electronic calculator, plotter, and any other item appropriate to the particular flight—for example, if a night flight is to be undertaken, carry a flashlight; if a flight is over desert country, carry a supply of water and other necessities.
WEATHER CHECK
It may be wise to check the weather before continuing with other aspects of flight planning to see, first of all, if the flight is feasible and, if it is, which route is best.
USE OF THE AIRPORT/FACILITY DIRECTORY
Study available information about each airport at which a landing is intended. This should include a study of the Notices to Airmen (NOTAMs) and the Airport/Facility Directory. This includes location, elevation, runway and lighting facilities, available services, availability of aeronautical advisory station frequency (UNICOM), types of fuel available (use to decide on refueling stops), AFSS/FSS located on the airport, control tower and ground control frequencies, traffic information, remarks, and other pertinent information. The NOTAMs, issued every 28 days, should be checked for additional information on hazardous conditions or changes that have been made since issuance of the Airport/Facility Directory.
The sectional chart bulletin subsection should be checked for major changes that have occurred since the last publication date of each sectional chart being used. Remember, the chart may be up to 6 months old. The effective date of the chart appears at the top of the front of the chart. The Airport/Facility Directory will generally have the latest information pertaining to such matters and should be used in preference to the information on the back of the chart, if there are differences.
AIRPLANE FLIGHT MANUAL OR PILOT'S OPERATING HANDBOOK
The Airplane Flight Manual or Pilot's Operating Handbook (AFM/POH) should be checked to determine the proper loading of the airplane (weight and balance data). The weight of the usable fuel and drainable oil aboard must be known. Also, check the weight of the passengers, the weight of all baggage to be carried, and the empty weight of the airplane to be sure that the total weight does not exceed the maximum allowable. The distribution of the load must be known to tell if the resulting center of gravity is within limits. Be sure to use the latest weight and balance information in the FAA-approved Airplane Flight Manual or other permanent airplane records, as appropriate, to obtain empty weight and empty weight center-of-gravity information.
Determine the takeoff and landing distances from the appropriate charts, based on the calulated load, elevation of the airport, and temperature; then compare these distances with the amount of runway available. Remember, the heavier the load and the higher the elevation, temperature, or humidity, the longer the takeoff roll and landing roll and the lower the rate of climb.
Check the fuel consumption charts to determine the rate of fuel consumption at the estimated flight altitude and power settings. Calculate the rate of fuel consumption, and then compare it with the estimated time for the flight so that refueling points along the route can be included in the plan.
RADIO NAVIGATION: VERY HIGH FREQUENCY (VHF) OMNIDIRECTIONAL RANGE (VOR)
There are four radio navigation systems available for use for VFR navigation. These are:
VHF Omnidirectional Range (VOR)
Nondirectional Radiobeacon (NDB)
Long Range Navigation (LORAN-C)
Global Positioning System (GPS)
VERY HIGH FREQUENCY (VHF) OMNIDIRECTIONAL RANGE (VOR)
The VOR system is present in three slightly different navigation aids (NAVAIDs): VOR, VOR/DME, and VORTAC. By itself it is known as a VOR, and it provides magnetic bearing information to and from the station. When DME is also installed with a VOR, the NAVAID is referred to as a VOR/DME. When military tactical air navigation (TACAN) equipment is installed with a VOR, the NAVAID is known as a VORTAC. DME is always an integral part of a VORTAC. Regardless of the type of NAVAID utilized (VOR, VOR/DME or VORTAC), the VOR indicator behaves the same. Unless otherwise noted, in this section, VOR, VOR/DME and VORTAC NAVAIDs will all be referred to hereafter as VORs.
The word "omni" means all, and an omnidirectional range is a VHF radio transmitting ground station that projects straight line courses (radials) from the station in all directions. From a top view, it can be visualized as being similar to the spokes from the hub of a wheel.
The distance VOR radials are projected depends upon the power output of the transmitter. The course or radials projected from the station are referenced to magnetic north. Therefore, a radial is defined as a line of magnetic bearing extending outward from the VOR station. Radials are identified by numbers beginning with 001, which is 1° east of magnetic north, and progress in sequence through all the degrees of a circle until reaching 360. To aid in orientation, a compass rose reference to magnetic north is superimposed on aeronautical charts at the station location.
VOR ground stations transmit within a VHF frequency band of 108.0 – 117.95 MHz. Because the equipment is VHF, the signals transmitted are subject to line-of-sight restrictions. Therefore, its range varies in direct proportion to the altitude of receiving equipment. Generally, the reception range of the signals at an altitude of 1,000 feet above ground level (AGL) is about 40 to 45 miles. This distance increases with altitude.
VORs and VORTACs are classed according to operational use. There are three classes:
T (Terminal)
L (Low altitude)
H (High altitude)
PRINCIPLES OF FLIGHT
Though there are various kinds of pressure, this discussion is mainly concerned with atmospheric pressure. It is one of the basic factors in weather changes, helps to lift the airplane, and actuates some of the important flight instruments in the airplane. These instruments are the altimeter, the airspeed indicator, the rate-of-climb indicator, and the manifold pressure gauge.
Though air is very light, it has mass and is affected by the attraction of gravity. Therefore, like any other substance, it has weight, and because of its weight, it has force. Since it is a fluid substance, this force is exerted equally in all directions, and its effect on bodies within the air is called pressure. Under standard conditions at sea level, the average pressure exerted on the human body by the weight of the atmosphere around it is approximately 14.7 lb./in. The density of air has significant effects on the airplane's capability. As air becomes less dense, it reduces (1) power because the engine takes in less air, (2) thrust because the propeller is less efficient in thin air, and (3) lift because the thin air exerts less force on the airfoils.
EFFECTS OF PRESSURE ON DENSITY
Since air is a gas, it can be compressed or expanded. When air is compressed, a greater amount of air can occupy a given volume. Conversely, when pressure on a given volume of air is decreased, the air expands and occupies a greater space. That is, the original column of air at a lower pressure contains a smaller mass of air. In other words, the density is decreased. In fact, density is directly proportional to pressure. If the pressure is doubled, the density is doubled, and if the pressure is lowered, so is the density. This statement is true, only at a constant temperature.